Enhanced Soil Carbon Management
Soil carbon sequestration (SCS) refers to long-term carbon storage in soil. Although SCS occurs naturally, it has been disrupted by human activity, particularly farming. Farmers can enhance SCS by adopting soil carbon management practices that add carbon back into the soil and/or avoid carbon loss. Typical practices include the following: livestock grazing management, cover cropping, organic and synthetic inputs, and tillage practices. Although enhanced soil carbon management is one of the only carbon dioxide removal practices that can already be deployed at large-scale, Giving Green does not recommend soil carbon offsets. It is challenging to measure whether soil carbon management practices are increasing stored carbon, and most soil carbon projects do not have a plan for ensuring permanence.
This report last updated April 19, 2022. Questions and comments are welcome.
Download the full report to access the appendix, detailing methods for measurement of changes in soil carbon, and the full list of works cited.
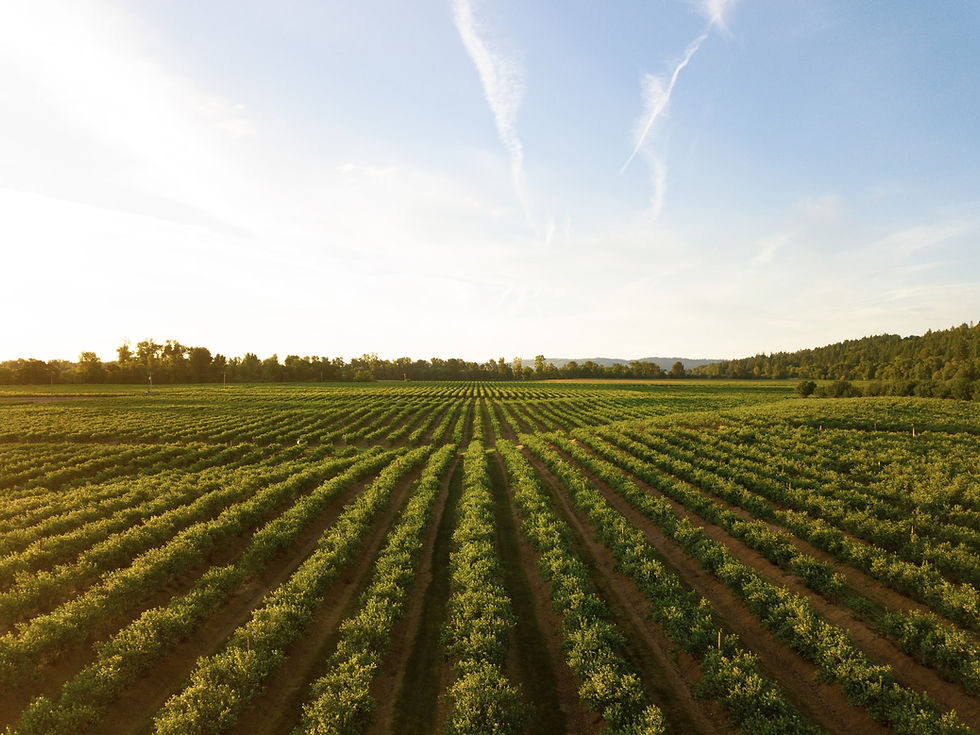
Image: Dan Meyers
What is soil carbon?
There are two types of soil carbon: soil organic carbon (SOC) and soil inorganic carbon (SIC).
Soil organic carbon – SOC is “composed of soil microbes including bacteria and fungi, decaying material from once-living organisms such as plant and animal tissues, fecal material, and products formed from their decomposition” (Ontl & Schulte, 2012). SOC levels depend on interactions between ecosystem processes such as photosynthesis, respiration, and decomposition. These processes are influenced by climatic conditions, especially soil temperature and soil moisture. For example, dry regions tend to have lower SOC levels than temperature and tropical regions.
Soil inorganic carbon – SIC, primarily found as carbonate minerals such as calcite and dolomite, is formed either through the wearing-away of rocks or from soil minerals reacting with atmospheric CO2.
How is carbon stored in soil, and how is it lost?
Soil gains and loses carbon as part of the carbon cycle, which involves the travel of carbon atoms between several different carbon pools (e.g., the Earth’s crust, the atmosphere, the biosphere). For example, carbon can enter the soil from the atmosphere when plants fix CO2 from the air and release fixed carbon into the soil via their roots. Carbon can also enter the soil when leaf and root litter and non-living microbial biomass become part of the soil. SOC leaves the soil when microorganisms break down organic carbon sources and release CO2 during cellular respiration. Although SIC is generally considered more stable as a carbon stock than SOC, it can still decrease due to agricultural practices that affect water flow, land use, and soil acidification (Raza et al., 2021).
What determines whether soil is a carbon source or sink?
Soil can either be a net sink or source of carbon, depending on the balance between the soil’s carbon inputs and outputs. This balance is influenced by factors such as types of above-ground plants present, types of substances released by plant roots, types of microorganisms present in the soil, and environmental variables (e.g., soil moisture, soil temperature, and nitrogen levels in the soil). Turning soil into a net sink of CO2 typically means increasing the amount of carbon input (e.g., increasing above- and below-ground biomass) and/or reducing carbon losses (e.g., restricting soil disturbance).
What accelerates carbon loss from soil?
Soil degradation has been accelerated by human activities, such as deforestation, overgrazing, and intensive agriculture (Lemus & Lal, 2005). These activities can affect carbon loss in various ways:
Disrupting the soil structure – Soil disturbances can increase soil erosion and run-off, transporting carbon-rich material away from a field (Starr et al., 1999).
Exposing soil carbon to oxidative processes – Practices such as tilling exposes soil carbon to oxygen and facilitates oxidation, releasing CO2 in the process.
Reducing plant roots and residues – Deforestation and overgrazing decrease how much plant roots and residues are in the soil. These activities reduce carbon inputs and negatively affect how much organic matter can accumulate in the soil (Jastrow, 1996).
Increasing temperature – Deforestation can raise soil temperature by changing solar radiation, wind speed, and air temperature (Hashimoto & Suzuki, 2004). Because microbial activity generally increases with soil temperature up to a point, increased temperatures can increase soil microorganisms’ rate of cellular respiration and how much CO2 they produce (Walker et al., 2018).
It is estimated that soil degradation due to agricultural land use has led to a loss of about 133 billion metric tons of carbon over the past 12,000 years, with carbon loss accelerating over the past 200 years (Sanderman et al., 2017).
How much CO2 can enhanced soil carbon management mitigate?
The Intergovernmental Panel on Climate Change (IPCC) has medium confidence that enhanced soil carbon management for croplands has a technical mitigation potential of 0.4 to 6.8 billion metric tons of CO2- equivalent per year (Pathak et al., 2022). This wide range may be representative of how much uncertainty there is over how much agriculture practices improve SCS. Its economic mitigation potential, or how much carbon can be sequestered at a cost less than or equal to $100 per ton of CO2-equivalent, is closer to the lower end of this range.
Enhanced soil carbon management as a carbon offset
Mechanism
Practices that improve SCS can either remove carbon and/or avoid emissions. For example, growing perennial crops instead of annual crops may increase carbon capture via photosynthesis throughout the year and reduce soil disturbances, which helps prevent carbon loss. For more information, please see Table 1.
The rate at which soil can sequester carbon decreases as the soil becomes saturated with carbon (e.g., carbon inputs become balanced with outputs) (Stewart et al., 2007). After a new agricultural practice is adopted to increase soil carbon storage, it may take decades before the soil reaches carbon saturation; the amount of time depends on the practice, soil type, and climate zone (Hatzell & Wilcox, 2021). The IPCC uses a default saturation time of 20 years. Stored carbon may be lost after SCS management is reversed (Figure 1). For example, going from no-till methods to conventional tillage would lead to carbon loss.
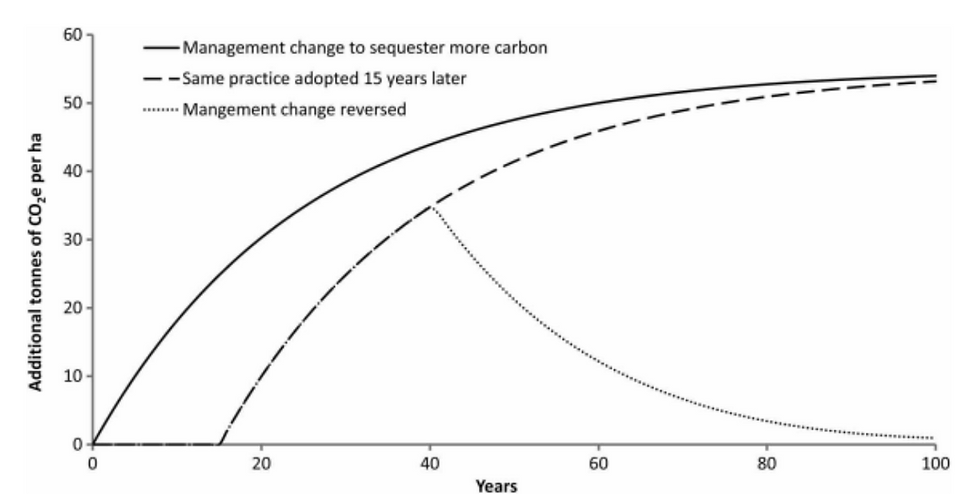
Figure 1: Stylized dynamics of carbon sequestration (Thamo & Pannell, 2016)
Causality
Soil carbon management practices that can improve SCS
Soil carbon management practices that can enhance SCS include but are not limited to the following:
Livestock grazing management: Livestock grazing can be rotated between pastures to stimulate plant regrowth and add manure to the soil, enhancing plant growth and soil productivity. Rotating livestock between fields also reduces soil compaction; compaction limits air and water permeability in the soil (Whalley et al., 1995) and can reduce root growth (Pandey et al., 2021). Primary mechanism(s) for increased SCS: Increased carbon input, Reduced carbon losses
Increasing the amount of time that plants remain in the ground: Cover crops are meant to cover the soil and are not meant for harvest. They may be grown outside of the primary growing season (e.g., winter instead of summer). Perennial crops are intended to be grown year-round. Cover crops and perennial crops protect soil from erosion and increase carbon inputs to the soil. Primary mechanism(s) for increased SCS: Increased carbon input, Reduced carbon losses
Organic and synthetic inputs: Inputs such as biochar (charcoal produced in the absence of oxygen), crop residues (plant materials left in a field after harvest), and fertilizer add nutrients and/or carbon to the soil. Inputs can enhance plant and root growth. Primary mechanism(s) for increased SCS: Increased carbon input
Tillage practices: Conservation tillage practices such as no-till and strip tillage are considered less intense than conventional tillage (UC Sustainable Agriculture Research and Education Program, 2017). These practices minimize soil disturbance and can lead to improved soil carbon retention. Leaving land unused for farming can also reduce soil erosion. Primary mechanism(s) for increased SCS: Reduced carbon losses
Non-agricultural practices that can improve SCS include forest management, peatland restoration, coastal wetland restoration, and grassland fire management.
Uncertainties related to causality
Enhanced soil carbon management can reduce levels of CO2 in the atmosphere, but it is unclear to what degree and for how long. Uncertainties related to causality include the following:
Dependence on local context – The degree to which soil carbon management practices can improve SCS depends on baseline practices, initial levels of SOC, and location-specific factors such as geographic, soil, and climatic conditions (Moore et al., 2021). These factors determine how much additional carbon can be stored in the soil and when the soil will reach its saturation point.
Challenges in measuring soil carbon accurately
It is challenging to separate the ‘signal’ of management effects on soil carbon from local ‘noise’ given that (1) the total amount of stored carbon in steady-state changes very slowly over time, (2) levels of SOC can vary significantly across a single field (Bradford et al., 2019) (3) weather can cause short-term fluctuations in COS. Additionally, the net addition of soil carbon per hectare is very small (Pathak et al., 2022).
Accurate direct measurements of SOC require sampling at high spatial density, which can be expensive and time-consuming.
Although soil crediting projects can rely on modeling instead of direct measurements to quantify soil carbon gains, this is probably less accurate given the various assumptions that the models must make.
Unknowns in soil science – There are still considerable unknowns in soil science. For example, few studies on SCS have included soil carbon samples taken at depths beyond 30 cm; it is possible that no-till practices have been overvalued given the lack of sampling at greater depths (Meurer et al., 2018).
Lack of differentiation between soil carbon management projects – It is unclear how the efficacy of different soil carbon management projects compare against one another. There needs to be further work on disaggregating the various practices that contribute to SCS (Meurer et al., 2018). It is essential to differentiate between the various methods because they use different mechanisms (e.g., increasing carbon input and/or reducing carbon loss) to increase stored carbon and vary in feasibility. For example, farmers may view some practices as more acceptable than others.
Potential increase in other greenhouse gases (GHGs) – Soil carbon management projects involving nitrogen fertilizers can increase nitrous oxide emissions if the fertilizer is not appropriately managed.
Potential for carbon leakage – Soil carbon management projects can lead to carbon leakage where increases in GHGs occur outside of project boundaries (Murray et al., 2007). For example, if farmers who practice no-till had lower corn yields, this decreased supply could increase corn prices and encourage other farmers to grow more corn using conventional tilling practices.
In general, causality is uncertain for soil carbon offsets. A project would need to have excellent data supporting causality for us to be confident in it.
Project additionality
It seems likely that many soil carbon management practices have project additionality, meaning they must be enabled by carbon offsets. Namely, there are enough upfront capital costs, operational costs, and other obstacles (e.g., access to new markets) that most farmers probably need financing to maintain these practices. Furthermore, these new agricultural practices would need to be maintained indefinitely to prevent stored carbon from being released. At the same time, however, some farmers have already adopted certain practices without any need for offsets given their co-benefits, such as potentially higher crop yields. This raises some questions as to these practices’ additionality if farmers are willing to adopt new practices without carbon offset credits. Finally, it is unclear how project additionality varies between different soil carbon management practices.
Marginal additionality
Marginal additionality is achieved if each soil carbon offset leads to additional GHG removal. SCS scores high on marginal additionality because SCS practices can be expanded to more and more farmers and land.
Permanence
SCS is impermanent. Because soil carbon loss depends on the balance between carbon inputs and outputs, sites can lose soil carbon naturally outside of farming practices (Murray et al., 2007). Severe droughts, for example, can make an environment inhospitable to plants and therefore reduce carbon inputs. Soil carbon loss can also occur after farmers stop soil carbon management practices and switch to conventional methods. Therefore, farmers would likely need to be paid to continue soil carbon management practices over the long term even after the soil has reached its saturation point to maintain gains in soil carbon storage.
Switching from soil carbon management practices to conventional methods is unlikely to lead to immediate carbon loss. For example, a synthesis report on periodic tillage found that a single tillage event is unlikely to eliminate carbon gains immediately (Conant et al., 2007). Instead, a single tillage event could lead to a decline in soil carbon of 1-11%, and losses increase as tillage frequency increases. Additionally, one study found that when farmyard manure was applied to a cereal cropping system for twenty years and then halted, the soil still contained about 2.5 times more soil organic matter (a source of soil carbon) 150 years later than soil that never received manure (Johnston et al., 2009, p. 1). Finally, decay kinetics predict that it would take at least several years for soil to lose all newly gained carbon (Schimel et al., 1994).
Co-benefits
Benefits to soil, plant, and ecological health
In addition to its climate benefits, soil carbon also provides multiple benefits to soil, plant, and ecological health (Milne et al., 2015). These benefits include the following:
Maintaining soil structure – Soil carbon helps maintain soil structure by forming larger groups of soil particles (aggregates). These larger aggregates increase the soil’s water storage capacity by creating larger pores between aggregates. Larger pore space also improves aeration and drainage.
Supporting microbial activity – Soil carbon provides substrate and energy for microorganisms. Microorganisms play a role in promoting plant growth by influencing root development (Verbon & Liberman, 2016), outcompeting harmful microorganisms (Mendes et al., 2013), and increasing the bioavailability of nutrients (van der Heijden et al., 2008).
Supporting plant productivity – Soil carbon can improve retention of organic nitrogen, phosphorus, and other nutrients that support plant productivity.
Resisting erosion – Soil carbon helps keep the soil more physically cohesive, which can help prevent erosion and have positive effects on both water quality and local ecology.
Benefits to farmers
Improvements to soil health due to improved SCS can potentially benefit farmers in numerous ways:
Increased crop yield – Because soil carbon improves soil health, adding one ton of carbon per hectare on degraded cropland soil can potentially increase crop yield by a range from 0.5 kg per hectare for cowpeas to 40 kg per hectare for wheat (Lal, 2004). However, the degree to which increased soil carbon impacts crop yield relies on the field’s existing soil health and crop type.
Improved climate resilience – Increased soil carbon content can make soil more resilient against droughts (Iizumi & Wagai, 2019) and heavy rainfall (Rabot et al., 2018).
Reduced need for fertilizer – Healthy soil can reduce farmers’ fertilizer needs, leading to cost savings and reduced environmental impact (Oldfield et al., 2019).
Negative co-benefits to farmers
Farmers may not want to adopt soil carbon management practices if they do not fit their preferences. Risks or setbacks related to these practices include the following (Marland et al., 2001):
Increased risk – Conventional tillage kills weeds by burying them. Less intensive tillage methods, which are better for SCS, may decrease crop yield by increasing the number of weeds.
More intensive management practices – Some practices may increase farmers’ workloads. Less intensive tillage methods, for example, may increase the amount of weeding that farmers need to do and/or lead to increased herbicide use. Additionally, rotating livestock between fields is more work than letting livestock graze in the same area.
Need for long-term commitment – Farmers may be unwilling to commit to soil carbon management practices over the long term. Furthermore, it is unclear how this liability would be passed on between farmers when farm ownership changes. Notably, nearly 40% of US farmland in 2012 was operated by renters (Amundson & Biardeau, 2018).
Cost-effectiveness
According to the literature on SCS, practices that enhance SCS can cost between -$45 to $100 per ton of CO2 (de Coninck et al., 2018); negative costs are associated with co-benefits such as improved productivity and resilience. There is a wide range of possible costs because SCS potential varies from place to place. For instance, degraded soils have a higher potential for soil carbon gain than healthier soils. Additionally, the costs of soil carbon management practices differ. The IPCC reports that land management for cropland and grazing land has a cost of $20 per ton of CO2-equivalent while restoring organic soils costs $100 per ton of CO2-equivalent (Nabuurs et al., 2022).
In 2021, Microsoft spent $2 million on soil carbon credits from Truterra/Land O’Lakes at a contracted volume of 100,000 metric tons of CO2 removed and a contracted durability of 20 years (Microsoft, 2021; Vasquez, 2021). The cost of this project, which focuses on science-based crop management, was $20 per ton of CO2. Using CarbonPlan’s permanence calculator, the cost of permanent CO2 removal ranges from $73 to $123 per metric ton when we assume a project duration of 20 years, discount rate of 3%, risk of project failure of 10% per year, and permanent cost of $500 per metric ton of CO2. It is unclear what agricultural practices are involved in this particular project; it may include some combination of cover crops, reduced tillage, and reduced usage of fertilizer and chemicals (Plume, 2021). Microsoft has also purchased soil carbon credits related to cattle grazing management at a contracted volume of almost 100,000 metric tons of CO2 removed, but this cost is not publicly available.
Giving Green’s assessment of SCS
We are concerned about SCS’s permanence and the high uncertainty over whether agricultural practices are improving SCS. In particular, it is challenging to measure changes in stored carbon over the long term accurately, and there are still open questions on what SCS practices are most effective and where. Therefore, although we view SCS’ co-benefits and additionality positively, we are generally skeptical about soil carbon credits overall. In order to be considered for our recommendation, a project would need especially good data on actual increase in soil sequestration, and a plan to maintain permanence.